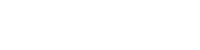
How Many Black Holes Are In The Solar System?
Season 10 Episode 31 | 18m 32sVideo has Closed Captions
Dark matter has eluded us for many decades but we may be able to discover more now.
Dark matter has eluded us for many decades. Even our most advanced particle colliders and sophisticated underground detectors have come up short. But it may be that we can finally solve this mystery with a much simpler experiment, involving a ray of light, a good clock, and the planet Mars.
Problems with Closed Captions? Closed Captioning Feedback
Problems with Closed Captions? Closed Captioning Feedback
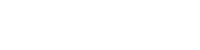
How Many Black Holes Are In The Solar System?
Season 10 Episode 31 | 18m 32sVideo has Closed Captions
Dark matter has eluded us for many decades. Even our most advanced particle colliders and sophisticated underground detectors have come up short. But it may be that we can finally solve this mystery with a much simpler experiment, involving a ray of light, a good clock, and the planet Mars.
Problems with Closed Captions? Closed Captioning Feedback
How to Watch PBS Space Time
PBS Space Time is available to stream on pbs.org and the free PBS App, available on iPhone, Apple TV, Android TV, Android smartphones, Amazon Fire TV, Amazon Fire Tablet, Roku, Samsung Smart TV, and Vizio.
Providing Support for PBS.org
Learn Moreabout PBS online sponsorshipDark matter has eluded us for many decades.
Even our most advanced particle colliders and sophisticated underground detectors have come up short.
But it may be that we can finally solve this mystery with a much simpler experiment, involving a ray of light, a good clock, and the planet Mars.
Over 80% of the mass in the universe is in the form of some mysterious, invisible, unseeable substance that, for want of any idea whatsoever what it is, we call dark matter.
At first, many of us thought that it was just some sort of regular stuff that wasn’t easy to see—maybe lots of small, dense, non-shining bodies like brown dwarfs or neutron stars or even black holes.
But as we ruled out most of the possible mass ranges for these, more and more of the dark matter hunting has been about finding some kind of exotic particle—Neutrinos, axions, etc.
But, again, after years and years of looking for the dark matter particle, we’re in the same place with this hypothesis—there’s just no credible evidence in favor of any hypothesis for what dark matter really is.
It’s like when you look for your keys in the usual spots, don’t find them so check under the couch, under the cat, inside the refrigerator.
But when you still don’t find them you double check your pockets.
Maybe it was in one of the obvious places all along.
And there are indeed a couple of pockets still to check, where “pockets” means range of masses for compact bodies.
The most promising under-explored range is 10^17 to 10^23 g, roughly the mass range of asteroids.
Now, dark matter can’t be asteroids because there’s no conceivable way to make 5 times the mass in asteroids than there is in stars—after all, asteroid material is made inside stars.
There aren’t that many stars, and also dark matter had to be around before the first stars.
But there is an option for a truly invisible object in that mass range, and one that would have been around since near the beginning and that's the primordial black hole (PBH).
These days, black holes are formed in the deaths of massive stars and their masses start at around 3 times the Sun’s mass.
But, as we’ve seen before on this show, there is a way to produce asteroid-mass black holes, and that’s right after the Big Bang.
Back then, there were tiny variations in the density of the hyper-dense sea of matter and energy that filled the universe.
Some of these would later collapse under gravity into galaxies and galaxy clusters, but right at the beginning the most dense such regions could have collapsed directly into black holes.
These primordial black holes could have a range of masses depending on yet-unknown details about the very early universe.
But if we tune our Big Bang parameters just right, we can get enough PHBs in the right mass range to account for all of dark matter.
Not only have we talked about PBHs before, we’ve talked about PBHs as dark matter, AND we’ve talked about the possibility of detecting PBHs from the scars they might leave as they pass through the Earth, or even the Moon.
But such a detection is going to take quite a bit of luck—the Earth and Moon make pretty small targets on the scale of the solar system, and distinguishing the scars from old PBH impacts isn’t necessarily so easy.
But it turns out that we may not even need PBHs to hit planets in order to detect them.
Recent studies have shown that our entire solar system may serve as a gigantic PBH detector, with these little black holes leaving their faint but very possibly detectable gravitational signature on the orbits of the planets.
Let’s run some numbers to see if this can really make this work.
And by “we” I mean the scientists who are actively figuring this out as we speak.
One paper I’ll focus on in particular Tung Tran, Sarah Geller, Benjamin Lehmann and David Kaiser and it was published just this September and I think does the best job at assessing the plausibility of turning the solar system into a black hole detector.
Let’s start with dark matter.
Based on the orbits of stars in the Milky Way, we have a pretty good idea of the distribution of dark matter in our galaxy.
The density of dark matter at our solar system’s position in the galaxy is about 7x10^-25 g/cm^-3 or about the equivalent of the mass of a proton in every sugar cube of volume.
So, if dark matter is a proton-mass particle, something like 10 million of them pass through you every second.
Experiments trying to detect dark matter particles assume that they stream through our detectors in enormous numbers, so that even if there’s a very low probability of interaction, we’ll eventually catch some.
Although apparently not yet.
The more massive the dark matter particle, the lower the number density.
If dark matter is a compact object like a black hole, then they’d be pretty spread out.
For example, if all dark matter consists of Sun-mass black holes, there’d need to be one per 15 cubic light year volume to do the job.
So what mass range might these PBHs have if they really do account for all of dark matter?
Astronomer’s favourite way to constrain has been with gravitational lensing.
Although black holes are invisible, their gravitational fields warp the paths of light rays passing close by.
If a black hole passes between us an a more distant star, that star will briefly brighten as its light gets focused towards us in an effect called microlensing.
The OGLE survey recently published new results from their decades-long effort to count compact objects in the Magellanic clouds this way.
They rule out huge mass ranges of primordial black holes as dark matter, everything from half the mass of the moon up to about 1% of the mass of the sun, roughly the boundary between brown dwarfs and proper stars- that’s a factor of a million in mass.
And in fact the only remaining mass window that’s still wide open to potentially account for all of dark matter is the so-called “asteroid mass range”, from around 10^17 grams to 10^23 grams, or less than 0.1% of the moon's mass.
If such small black holes make up all dark matter we’re going to need a lot of them.
If PBHs are at the bottom of range—10^17 grams—then we’d expect there to be one somewhere in the inner solar system right now.
At the upper end of this range of asteroid-mass black holes, we might expect one to pass through the solar system every century.
In between these extremes we have them passing through the solar system on timescales of months to decades.
And that is frequent enough that we should think about how to detect one when it comes.
So we’re not going to see a primordial black hole with a telescope.
In the asteroid mass range, our PBHs have event horizons that range from the subatomic to the microscopic.
So even if they were visible they wouldn’t be visible.
Instead, we’d spot a PBH passing through our solar system in the same way we discovered dark matter in the first place—from its gravitational influence.
When an interstellar object enters the solar system it almost always exits again—the speed it gathers falling into the Sun’s gravitational field is, by definition, exactly what it needs to escape.
Add to this the fact that an interstellar object is on a different orbit to the solar system, so it’s already coming in hot—with some appreciable fraction of the galactic orbital speed of 220 km/s.
The result is that a black hole fly-through is going to be pretty quick, with a passage through the inner solar system lasting a few months at most.
If this is the sort of black hole produced by the death of a star—a stellar black hole—then its effect on the planetary system would be devastating.
Orbits may be significantly altered, planets ejected, and the black hole may even capture planets into its own orbit.
However, the effect of an asteroid-mass primordial black hole would be no more significant than an actual interstellar asteroid passing through the solar system—which means almost no effect.
Planets are many millions to trillions of times more massive than any such PBH, and so the acceleration a planet experiences due to that passage will be commensurately tiny.
But tiny isn’t zero.
Think about it this way: imagine you and another car are side by side on the highway both going exactly 100.00 km/hr.
Your cruise control glitches and you speed up to 100.01 km/hr.
You don't notice that right away, but an hour later your car will be 10 meters ahead, that’s an entire car length, which you’ll definitely notice.
In the same way, a tiny, parts-per-trillion change in a planet’s position will be visible over years.
Let’s say a PBH in the upper-middlish of our asteroid-mass range—10^21 grams—passes through our solar system a little way outside the orbit of Mars.
The red planet’s change in speed would be pretty much unmeasurable at the time.
But after a decade it would add up to a difference in position of about 1m compared to the expected location.
That also sounds pretty hard to measure, given the 10s of millions of km distance between Earth and Mars.
But there are two factors that make this actually possible.
The first is that the motion of the planets is predictable.
With clockwork precision, the planets are slaves to the law of gravity—whether Newton’s or Einstein’s.
If we know the positions and velocities of a system of gravitating bodies at one point in time, we can trace their motion far into the future.
Neptune was discovered based on a small discrepancy in the motion of Uranus, and that was calculated with pen and paper back in the middle 1800s.
Modern computers can compute the future motion of the planets with incredible precision, although including the gravitational influence of the many smaller residents of the solar system starts to make it complicated, and I’ll come back to that.
Knowing precisely where a planet is, even down to the centimeter, isn’t helpful if we can’t actually measure the distance to the planet with that same precision.
But fortunately, that’s also within humanity’s skill set.
We don’t measure distances to planets directly.
Instead, we measure the travel time of light.
Conveniently, the speed of light in the near vacuum of interplanetary space is constant and extremely well known—so timing its round trip from Earth and back gives us a distance that’s as precise as the stopwatch we use to time that trip.
And we do have some exceedingly precise atomic stopwatches these days.
One of the most precisely measured distances in the solar system is the Earth-Moon separation.
It’s known to about 1 mm precision thanks to the retroreflectors—fancy mirrors—placed on the lunar surface by the US, the Soviets, and now India.
The precision of our measurement of the Earth-Moon distance makes the moon sound like a good option for a good black hole detector.
But it’s actually not our best option.
The fact that the moon is so close means we can't really treat it as a single point.
But the Earth and Moon are big, slightly irregular balls that squish and stretch in each others gravity.
Although we can measure the distance to those retroreflectors with amazing precision it's hard to convert that to a well defined distance to the moon.
The trick then is to look farther, to Mars.
While Mars doesn’t yet have a retroreflector big enough this, we have something that may be even better--our own satellites orbiting Mars.
At any one time over the past 20 years, we have had at least three different functioning spacecraft in Martian orbit.
It's straightforward enough to get an exact distance to any one of these based on the signal travel time, and the satellites can use complex triangulation to get their own altitude above the Martian surface.
By collecting these distances over time and for multiple spacecraft, we can now define and keep track of a robust "distance to Mars", and have been able to do so for 20 years.
We can likely spot the 1 meter offset caused by the 10^21g PBH we saw earlier, and perhaps even the 10cm-ish offset caused by a PBH 10 times smaller.
That covers half of the possible range for PBH masses.
OK, so apparently we can both predict and measure the location of Mars with the precision needed to tell if it strayed by a foot or two from where it was supposed to be.
How do we know that was caused by a passing black hole?
The solar system is a busy place, packed with planets, moons, dwarf planets, asteroids, and comets.
The mini-tweaks that the Earth and Mars might get from a PBH is one of millions, all of which must be accounted for if the signal from the black hole is to be separated from the noise.
In fact, any asteroid in the inner solar system with a mass comparable to our PBH would need to be included in calculations, otherwise its gravitational effects will completely wash out the effect we’re searching for.
The good news is that, while 10^20 grams or so makes a black hole as small as an atom, it makes an asteroid of that mass about a 100 km across, and all asteroids that big are all pretty well tracked.
Also, a PBH flyby will have a very different trajectory to a solar system asteroid.
Objects in the solar system orbit pretty close to the same plane that the planets orbit in, and all at speeds low enough to keep them bound in the Sun’s gravitational field.
A passing PBH will be moving quite a bit faster, and could come in at any angle relative to the plane of the solar system.
In-plane flybys will tend to tweak a planet’s speed, but keep it in the same plane.
Steeper flybys will tend to tug a planet slightly out of that plane, altering its orbital inclination.
Because of this, the change in a planet’s position due to an extrasolar object versus a solar system object may be quite distinct.
Let’s talk about the two experiments we can do.
One we can do right now, and one is for the future.
We currently have around 20 years of precision data on the position of Mars, and in principle we could find the signs of past PBH passes in these data.
Some preliminary efforts have been made to analyze this, but not with conclusive results because it’s going to require some very sophisticated simulations to analyze the data properly.
The proper way to do this is by running many, many computer simulations to map the space of possible ways in which the orbit of Mars could evolve in response to the gravitational influence of known and unknown solar system objects.
That will tell us whether, at some time in the past 30 years, Mars strayed from this space of probable orbits in a way consistent with an interaction with a massive flyby.
Then we add potential extrasolar visitors to our simulation and see how well Mars’ trajectory is explained by different flyby masses and trajectories.
There’s no direct way to tell if such a flyby was really a PBH or just an extra-solar asteroid of the same mass.
If we find it in this old data then by now it’ll be long gone, so we can’t look for it with our telescopes.
We do know that there are such asteroids living between the stars—for example, Oumuamua, although it’s way below our desired mass range.
However, if dark matter is made of primordial black holes at these masses then those PBHs need to be way, way more abundant that extra-solar asteroids.
So if we find way too many such kicks to the Martian orbit in those 20 years of data, it’s good evidence that they’re coming from PHBs and not asteroids.
OK, so that’s the experiment we can do now, and astronomers are indeed working on this.
But what we’d really want is to get more direct evidence.
Monitoring future data from Mars can allow us to notice a change in its motion within a few years if we’re lucky, and then it might be possible to track the offending source of gravity as it coasts away from the solar system.
If it’s an asteroid then it’ll be visible to a sufficiently giant telescope.
If it’s a black hole it will not be.
So maybe we’ll discover what dark matter is by observing nothing.
It may be that to find the next level of action in particle physics we'll need to build a particle collider the size of the solar system.
Well, we already have a solar system sized particle detector that could discover dark matter.
Assuming dark matter is made of countless subatomic-sized black holes with the masses of asteroids.
In which case we can solve dark matter from miniscule shifts they cause in planetary paths through spacetime.